Unit 1
Thermodynamics
System
Whenever a change is to be analysed it is essential to specify the region in which the change take place. This is done by drawing a boundary aroundthe region. Boundary may be real or imaginary.
Everything within the boundary is called the system. The region outside the boundary is called surroundings
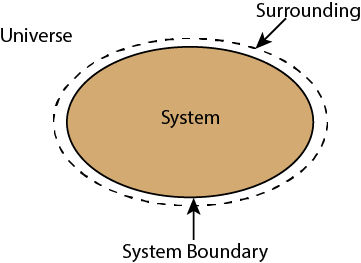
There are three types of Thermodynamics systems:
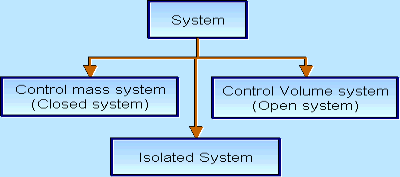
1 – Open system(control volume system): If mass and energy both cross the boundary of a system it is called open system .in open system both mass and energy can transfer across the boundaries and the mass within the system may not be constant .most of the engineering devices are open systems involving flow of fluids through them.
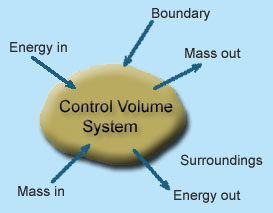
Example of open system
- Boiler – In boiler water convert from liquid to vapour phase. So mass and energy both transfer the boundary.
- Hydraulic turbine wheel – In this device potential energy of water convert into mechanical works. Water enters the wheel from head race side and leaves it to the tail race from the other end, and as such mass cross the system boundary.
- Motor-car engine – The engine sucks charge which is mixture of air and petrol and finally exhausts the gases to the surrounding atmosphere.
2 – Closed system (control mass system): If the mass within the boundary of the system does not change, it is called closed system. A closed system does not allow any mass transfer across the boundary but allow transfer of energy across the boundary.
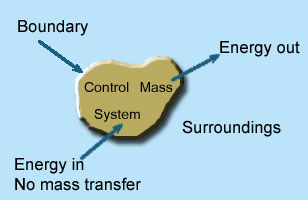
Example of closed system
- Piston cylinder assembly –the gas can reject or receive heat, expand or contract but no mass cross the boundary of system.
- Ignition system – electric energy cross the boundary to cause a spark between electrodes an initiate combustion .there is heat transfer but not mass across the system.
Pressure cooker, refrigerator, ice cream freezer etc. are the example of closed system.
3 - Isolated system: Neither mass nor energy cross the boundary of a system .it is called an isolated system. In this no mass and energy cross the system
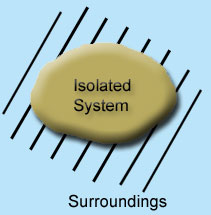
Example of isolated system
- Universe can be considered as an isolated system
- Fluid enclosed in a perfectly insulated closed vessel (thermos flask)
State:
The application of thermodynamic principles begins by defining a system that is in some sense distinct from its surroundings. For example, the system could be a sample of gas inside a cylinder with a movable piston, an entire steam engine, a marathon runner, the planet Earth, a neutron star, a black hole, or even the entire universe. In general, systems are free to exchange heat, work, and other forms of energy with their surroundings.
A system’s condition at any given time is called its thermodynamic state. For a gas in a cylinder with a movable piston, the state of the system is identified by the temperature, pressure, and volume of the gas. These properties are characteristic parameters that have definite values at each state and are independent of the way in which the system arrived at that state. In other words, any change in value of a property depends only on the initial and final states of the system, not on the path followed by the system from one state to another. Such properties are called state functions. In contrast, the work done as the piston moves and the gas expands and the heat the gas absorbs from its surroundings depends on the detailed way in which the expansion occurs.
The behaviour of a complex thermodynamic system, such as Earth’s atmosphere, can be understood by first applying the principles of states and properties to its component part in this case, water, water vapour, and the various gases making up the atmosphere. By isolating samples of material whose states and properties can be controlled and manipulated, properties and their interrelations can be studied as the system changes from state to state.
Path:
If all the change of states of system are plotted and all the points are conned, then the line joining the change of states of the system is called the path.
Thermodynamic equilibrium
Condition or state of a thermodynamic system, the properties of which do not change with time and that can be changed to another condition only at the expense of effects on other systems. For a thermodynamic equilibrium system with given energy, the entropy is greater than that of any other state with the same energy. For a thermodynamic equilibrium state with given pressure and temperature the Gibbs free energy is smaller than that of any other state with the same pressure and temperature.
Process:
Thermodynamics process represents a transition in which a system changes from one state to another. When the path is completely specified then the change of state is called a process. A Process is defined as the transformation of the system from one fixed state to another fixed state .When any one of the properties changes, the working substance or system is said to have undergone a process.
Some of the processes are identified by special names as given below:
- Isobaric process (constant pressure process)
- Isochoric process (constant volume process)
- Isothermal process (constant temperature process)
- Isentropic process (constant entropy process)
- Adiabatic process(perfectly insulated process)
If two systems are each in thermal equilibrium with the third system then the two systems are in thermal equilibrium which each other.
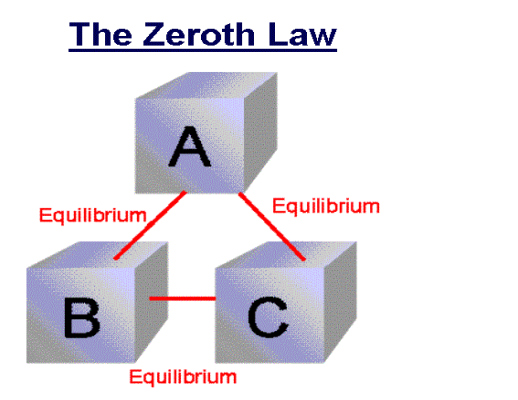
When a body A is in thermal equilibrium with a body B , and also separately with a body C ,then B and C will thermal equilibrium with each other .this is known as the Zeroth law of thermodynamics.
We use the zeroth law when we wish to compare the temperatures of two objects, A and B. We can do this by using a thermometer, C and placing it again object A it reaches thermal equilibrium with object A and measure the temperature of A. Placing the thermometer against object B until thermal equilibrium is reached we measure the temperature of object B. If they are the same temperature then they will be in thermal equilibrium with each other.
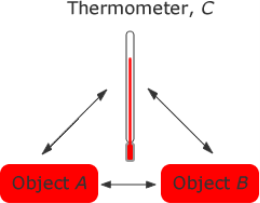
Figure 1. The Zeroth law of thermodynamics
First law of Thermodynamics
Sometimes our body start to sweat and feel warm when we are in a room full of people and the sweating becomes excessive if the room size is small. This happens because our body is trying to cool off hence heat transfers from our body in the form of ‘sweat’. This entails the first law of thermodynamics.
The first law of thermodynamics states that the total energy of an isolated system is constant. Energy can be transformed from one form to another, but can neither be created nor destroyed.
According to this law, some of the heat given to system is used to change the internal energy while the rest in doing work by the system. Mathematically,
ΔQ=ΔU+ΔW
Where,
ΔQ = Heat supplied to the system
ΔW= Work done by the system.
ΔU = Change in the internal energy of the system.
If Q is positive, then there is a net heat transfer into the system, if W is positive, then there is work done by the system. So positive Q adds energy to the system and positive W takes energy from the system.
It can also be represented as ΔU=ΔQ−W
We can say that internal energy tends to increase when heat is given to the system and vice versa.
Limitations of First Law of Thermodynamics
- The limitation of the first law of thermodynamics is that it does not say anything about the direction of flow of heat.
- It does not say anything whether the process is a spontaneous process or not.
- The reverse process is not possible. In actual practice, the heat doesn’t convert completely into work.If it would have been possible to convert the whole heat into work, then we could drive ships across the ocean by extracting heat from the water of the ocean.
Internal energy
Internal energy is the sum of the kinetic energies and the potential energies of the molecules. We denote the internal energy of the system by U. Energy possessed by the atoms or molecules by virtue of their motion is kinetic energy. Since there is a force of attraction between two molecules, they possess some potential energy. The sum of total kinetic and potential energies of atoms or molecules constituting a system is the internal energy of the system.The internal energy of a system depends upon the state of the system. It has a definite value for a definite thermodynamic state. It is not a measurable quantity. If a system starting from one state passes through different states and finally returns to its initial state, then the change in its internal energy will be zero.
Enthalpy
When a process takes place at constant pressure, the heat absorbed or released is equal to the Enthalpy change. Enthalpy is sometimes known as “heat content”, but “enthalpy” is an interesting and unusual word, so most people like to use it. Etymologically, the word “entropy” is derived from the Greek, meaning “turning” and “enthalpy” is derived from the Greek meaning “warming”. As for pronunciation, Entropy is usually stressed on its first syllable, while enthalpy is usually stressed on the second.
Enthalpy(H) is the sum of the internal energy(U) and the product of pressure(P) and volume(V).
Enthalpy H can be written as,
H = U + pV
Where, H = Enthalpy of the system
U = Internal energy of the system
p = Pressure of the system
V = Volume of the system
Enthalpy is not measured directly, however, the change in enthalpy (ΔH) is measured, which is the heat added or lost by the system. It is entirely dependent on the state functions T, p and U.
Enthalpy can also be written as
ΔH=ΔU+ΔPV
At constant temperature, for the process heat flow(q) is equal to the change in enthalpy, this is represented as
ΔH=q
Enthalpy Units
The Enthalpy is expressed as,
H = Energy/Mass
Any physical quantity can be represented by dimensions. The arbitrary magnitudes allocated to the dimensions are called units. There are two types of dimensions namely primary or fundamental and secondary or derived dimensions.
Primary dimensions are: mass, m; length, L; time, t; temperature, T
Secondary dimensions are the ones that can be derived from primary dimensions such as velocity(m/s2), pressure (Pa = kg/m.s2).
There are two unit systems currently available SI (International System) and USCS (United States Customary System) or English system. We, however, will use SI units exclusively in this course. The SI units are based on the decimal relationship between units.
Work
The scientific definition of work is different in many ways from its everyday meaning. The definition of work in physics reveals its relationship to energy – whenever work is done, energy is transferred.
For a work to be done, in a scientific sense, a force must be exerted and there must be displacement in the direction of the force. With this said, we can say that
Work is the product of the component of the force in the direction of the displacement and the magnitude of this displacement.
Mathematically, the above statement is expressed as follows:
W = (F cosθ) d = F. d
Where,
W is the work done by the force.
F is the force, d is the displacement caused by the force
Θ is the angle between the force vector and the displacement vector
The dimension of work is the same as that of energy and is given as = [ML2T–2].
Unit of Work
The SI unit of work is the joule (J), which is defined as the work done by a force of 1 Newton in moving an object through a distance of 1 meter in the direction of the force.
A weightlifter lifts a barbell weighing 25 kg and displaces it from the ground by 2 m. Here, the work done upon the barbell is against gravity.
The work done upon the weight against gravity can be calculated as follows:
Work done = (Mass × acceleration due to gravity) × Displacement
= (25 × 9.8) × 2 J
Heat
Heat is the transfer of kinetic energy from one medium or object to another or from an energy source to a medium or object. Such energy transfer can occur in three ways: radiation, conduction, and convection.
The standard unit of heat in the International System of Units (SI) is the calorie (cal), which is the amount of energy transfer required to raise the temperature of one gram of pure liquid water by one degree Celsius, provided the water temperature is higher than the freezing point and lower than the boiling point. Sometimes the kilocalorie (kcal) is specified as a unit of heat; 1 kcal = 1000 cal. (This is the so-called diet calorie.) Less often, the British thermal unit (Btu) is used. This is the amount of heat required to raise the temperature of one pound of pure liquid water by one degree Fahrenheit.
An example of heat by radiation is the effect of infrared (IR) energy as it strikes a surface. IR is an electromagnetic field capable of transferring energy from a source, such as a fireplace, to a destination, such as the surfaces within a room. Radiation does not require an intervening medium; it can occur through a vacuum. It is responsible for the warming of the Earth by the sun.
Heat by conduction takes place when two material media or objects are in direct contact, and the temperature of one is higher than the temperature of the other. The temperatures tend to equalize; thus the heat conduction consists of a transfer of kinetic energy from the warmer medium to the cooler one. An example is the immersion of a chilled human body in a hot bath.
Heat by convection occurs when the motion of a liquid or gas carries energy from a warmer region to a cooler region. A good example of convection is the tendency of warm air to rise and cool air to fall, equalizing the air temperature inside a room containing a hot stove. Heat convection (along with conduction) is believed to take place inside the Earth, transferring kinetic energy from the inner core through the outer core and mantle to the crust. In this situation, the outer core and the mantle behave like liquids over long periods of time.
Constant volume (Isochoric process):
Suppose a gas enclosed in a rigid vessel is interacting with the surroundings and absorbs energy Q as heat. Since the vessel is rigid, the work done W due to expansion or compression is zero. Applying the first law, we get
DU = dQ or Q = U2 –U1
That is, heat interaction is equal to the change in internal energy of the gas. If the system contains a mass m equal of an ideal gas, then
Q = ΔU = mCv (T2 –T1)
The path followed by the gas is shown on a P-V diagram. Now consider the fluid contained in a rigid vessel as shown. The vessel is rigid and insulated. Shaft work is done on the system by a paddle wheel as shown in Fig. a. In Fig. b electric work is done on the system. Since the vessel is rigid, the PdV work is zero. Moreover, the vessel is insulated and hence dQ = 0. Application of the first law of thermodynamics gives
DU = dQ – dW = dQ – (dWpdv + dWs)
OrdU = -dWs or – Ws = ΔU =U2 –U1
Where dWpdvis the compression /expansion work and dWs is the shaft work. That is increase in internal energy of a system at a constant volume, which is enclosed by an adiabatic wall, is equal to the shaft work done on the system.
Constant pressure (Isobaric process):
Several industrial processes are carried out at constant pressure. A few examples of constant pressure processes are: (a) reversible heating/cooling of a gas (b) phase change (c) paddle wheel work (d) electrical work. For a constant pressure process, the work done W is given by
W = ∫PdV = P (V2-V1)
Application of the first law of thermodynamics gives
DU = dQ – dW
= dQ – PdV
= dQ – d (PV)
Or dQ = dU + d (PV) = d(U + PV) = dH
Or Q = ΔH
That is in a constant pressure process, the heat interaction is equal to the increase in the enthalpy of the system. Now consider the constant pressure processes in which the system is enclosed by an adiabatic boundary. Application of the first law gives:
DU = dQ – dW = dQ – (PdV + dWs)
Here, the net work done (dW) consists of two parts – the PdV work associated with the motion of the boundary and (-dWs), the shaft work (or electrical work) done by the surroundings. Since the system is enclosed by an adiabatic boundary, dQ = 0 the equation can be written as
-dWs = dU + d (PV) = dH
That is, the increase in the enthalpy of the system is equal to the shaft work done on the system.
Constant temperature
Suppose a gas enclosed in the piston cylinder assembly is allowed to expand from P1 to P2 while the temperature is held constant. Then application of the first law gives:
DU = dQ – dW = dQ –PdV
It is not possible to calculate work and heat interactions unless the relationships between the thermodynamic properties of the gas are known. Suppose the gas under consideration is an ideal gas (which follows the relation Pv = RT and u =u (T) only) then for an isothermal process,
DU = 0
DQ = PdV = R.T.dv /v or Q =W = RTln(v2/v1)
Adiabatic process:
Reversible adiabatic process is also called an Isentropic Process. It is an idealized thermodynamic process that is adiabatic and in which the work transfers of the system are frictionless; there is no transfer of heat or of matter and the process is reversible. Such an idealized process is useful in engineering as a model of and basis of comparison for real processes.
Adiabatic process derivation
The adiabatic process can be derived from the first law of thermodynamics relating to the change in internal energy dU to the work dW done by the system and the heat dQ added to it.
DU =dQ-dW
DQ =0 by definition
Therefore, 0=dQ=dU +dW
The word done dW for the change in volume V by dV is given as PdV.
The first term is specific heat which is defined as the heat added per unit temperature change per mole of a substance. The heat that is added increases the internal energy U such that it justifies the definition of specific heat at constant volume is given as:
Cv =dUdT1n
Where,
N=number of moles
Therefore, 0= nCvdT+PdV …..(eq.1)
From the ideal gas law, we have
NRT =PV …..(eq.2)
Therefore, nRdT = PdV+VdP…. (eq.3)
By combining the equation 1. And equation 2, we get
−PdV= nCvdT = Cv / R (PdV+VdP)
0 = (1+Cv / R) PdV+Cv / RVdP
0= R+ Cv / Cv (dV / V) +dP /P
When the heat is added at constant pressure Cp, we have
Cp=Cv +R
0=γ (dV / V)+dP / P
Where the specific heat ɣ is given as:
γ≡Cp /Cv
From calculus, we have,
d (lnx)=dx / x
0=γd (lnV) + d (lnP)
0=d (γlnV+lnP) =d (lnPVγ)
PVγ=constant
Hence, the equation is true for an adiabatic process in an ideal gas.
Polytrophic process:
The term "polytropic" was originally coined to describe any reversible process on any open or closed system of gas or vapour which involves both heat and work transfer, such that a specified combination of properties were maintained constant throughout the process. In such a process, the expression relating the properties of the system throughout the process is called the polytropic path.
There are an infinite number of reversible polytropic paths between two given states; the most commonly used polytropic path is
T (dS/dT) = C1…..i
Where T is Temperature, S is Entropy, and C1 is a constant and is equal to zero for an adiabatic process. This path is equivalent to the assumption that the same amount of heat is transferred to the system in each equal temperature increment. In a reversible process following this polytropic path the heat and work transfer are as follows:
Q = C1 (T2-T1)…ii
And W = (H2-H1)-Q….iii
Where H is Enthalpy.
Pressure and volume, and pressure and temperature are related by the following expressions:
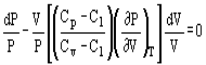
And
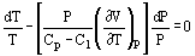
Where Cp and Cv are heat capacity at constant pressure and volume, respectively.
For an ideal gas this polytrophic path simplifies to
P.Vn=C2
Where C2 and n are constants and n is called the polytrophic index. The polytrophic index characterizes the process, as summarized and is given by
n = (Cp-C1) / (Cv-C1)
The equivalent pressure temperature and work relationships are as follows:
P (n-1)/n /T = C3 (NR/C21/n)
And
W=n/n-1 (P2V2-P1V1)
Many gas compression and expansion processes may be usefully approximated by a polytropic process. In each case the polytropic coefficient must be determined experimentally by measurement of the heat and work transfer and the initial and final states.
Belt, Rowlinson, and Saville (1975) discuss the errors and inconsistencies which may arise when PVn = C2 is applied to non-ideal gases and vapours.
The second law of thermodynamics can be understood by following two statements.
Kelvin-Planck statement
It is impossible to convert all the heat extracted from a hot body into work. In the heat engine, the working substance takes heat from the hot body, converts a part of it into work and gives the rest to the cold body. There is no engine that can convert all the heat taken from the source into work, without giving any heat into the sink. This means that for obtaining continuous work, a sink is necessary.
Clausius statement
It is not at all possible to transfer heat from a cold body to a hot body without the expenditure ofwork by an external energy source or its states that the heat energy cannot transfer from a body at a lower temperature to a body at a higher temperature without the addition of energy.
For example- There is a refrigerator that transfers an amount of heat from a cold body to a hot body without having any supply of external energy. So this is the violation ofClausius statement. Now suppose an engine working between the same hot and cold bodies takes in heat from hot body converts a part W into work and gives the remaining heat to the cold body.
The engine alone does not violate the second law of thermodynamics. But if the engine and refrigerator combine together, they form a device that takes up all the heat from the hot body and converts all into work without giving up any amount to the cold body. It violets the Kelvin-Planck statement. So we say that the two statements of the second law of thermodynamics are equal in all respects
Carnot cycle, in heat engines, ideal cyclical sequence of changes of pressures and temperatures of a fluid, such as a gas used in an engine. It is used as a standard of performance of all heat engines operating between a high and a low temperature.
In the cycle the working substance of the engine undergoes four successive changes: expansion by heating at a constant high temperature; reversible adiabatic expansion; compression by cooling at a constant low temperature; and reversible adiabatic compression. The engine receives heat (from the heat source) during the expansion at high temperature, delivers work during the reversible adiabatic expansion, rejects heat (to the heat sink) during the compression at low temperature, and receives work during the reversible adiabatic compression. The ratio of the network output to the heat input is equal to the ratio of the difference between the temperatures of the heat source and the heat sink divided by the temperature of the heat source. It represents Carnot’s principle in that it is the largest such ratio of any engine operating between the two temperatures.
The Carnot cycle consists of the following four processes:
- A reversible isothermal gas expansion process. In this process, the ideal gas in the system absorbs qin amount heat from a heat source at a high temperature Thigh, expands and does work on surroundings.
- A reversible adiabatic gas expansion process. In this process, the system is thermally insulated. The gas continues to expand and do work on surroundings, which causes the system to cool to a lower temperature, Tlow.
- A reversible isothermal gas compression process. In this process, surroundings do work to the gas at Tlow, and causes a loss of heat, qout.
- A reversible adiabatic gas compression process. In this process, the system is thermally insulated. Surroundings continue to do work to the gas, which causes the temperature to rise back to Thigh.
Figure 11: An ideal gas-piston model of the Carnot cycle.
P-V Diagram
The P-V diagram of the Carnot cycle is shown in Figure. In isothermal processes I and III, ∆U=0 because ∆T=0. In adiabatic processes II and IV, q=0.
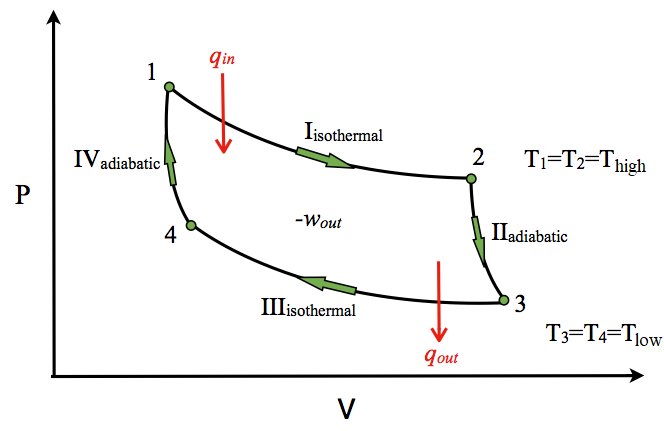
Question A Reverse Carnot cycle for refrigeration absorbs heat at 270K and rejects at 300K. Calculate the Coefficient of performance of this cycle .If the cycle is absorbing 1130kj/min at 270 K. How many kJ work is required per second?
Answer:
T1 = 270 K and T2 = 300 K
As we know that
C.O.P = T1/ T2 – T1
C.O.P = 270 / 300 – 270
C.O.P = 9
Work Require per second
Heat absorbed at 270 K,
Q1 = 1130 kJ/min = 18.83 kJ/s.
As we have discussed above
C.O.P = Heat Absorbed / Work Done
9 = 18.83 / Work Done
Work Done = 2.1 kJ / s
When we provide continuous heat to water then at 100 temperatures and 1 atm pressure, it boils and changes its phase from liquid to vapour. This vapour is known as steam.Steam contains more energy as it has both sensible heat and latent heat of vaporization. Steam has been a popular mode of conveying energy since the industrial revolution. Steam issued for generating power and also used in process industries such as sugar, paper, fertilizer, refineries, petrochemicals, chemical, food, synthetic fibre and textiles The following characteristics of steam make it so popular and useful to the industry:
• Highest specific heat and latent heat
• Highest heat transfer coefficient
• Easy to control and distribute
• Cheap and inert
Types: Wet steam, Dry steam, Superheated steam
Wet Steam: When steam contains water particles then it is known as Wet steam
Dry Steam: When wet steam is further heated then all water particles get converted into vapour and resulted steam is called dry steam.
Superheated Steam: When dry saturated steam is heated to higher temperatures then steam obtained is in superheated state. This steam is mostly used in Power generation.
Use :
(1) Power generation
(2) Heat engines (i.e. steam engines train)
Formation of steam at constant pressure:
Before going into formation of steam let we understand why steam is being formed/produced at constant pressure.The boiling temperature is 100 at one particular pressure that is 1 atm. Now if we change the pressure then the boiling temperature also changes. Since both are directly proportional then if we decrease pressure, boiling temperature decreases and vice versa.
After having understood the temperature and pressure fundamentals, let we discuss how we are going to produce steam from 1 kg piece of ice.Suppose the initial temperature of ice is a solid phase of water is -10.Now, keeping pressure constant which is again assumed as 1 atm, we provide continuous heat to the ice.
The graph is plotted between T and h. Let’s start with point A at which ice is at temperature -10. Now, as we provide heat to the piece of ice, its temperature as well as enthalpy increases simultaneously up to point ‘B’.
Point B is at 0 at which the phase transformation of solid ice into liquid takes place. This point is called melting point and temperature will remain constant until the whole transformation process is completed i.e all ice will convert in liquid at 0. This is so because of latent heat of fusion. All the heat we are providing is actually increasing the energy of the molecules of the water not its temperature, that’s why enthalpy increases but the temperature remains constant up to point C. Heat provided from point ‘B’ to point ‘C’ is hidden and cannot be measured using thermometer.
Since we are providing continuous heat the temperature and the enthalpy both increases (sensible heating). At ‘D’ which is boiling point of water. The next phase transformation i.e. liquid to vapour (steam) takes place. Since, the temperature again remains constant and enthalpy keep increasing, the heat absorbed during this is latent heat of vaporization. This process goes upto ‘E’. At ‘E’ we have converted all liquid into steam. If we keep providing heat then ultimately at ‘F’ superheated steam is obtained.
Thermodynamic properties of steam:
It is necessary to understand the properties of steam so that we can make proper use of it. Equipment size can be decided, pipe systems can be perfectly designed. It also allows us to make informed decisions affecting the energy usage of the system.
The properties are:
(1) Quality of steam (Dryness fraction)
Dryness fraction in simple words denotes the mass of dry steam in given steam. Or how much steam is dry or in other words how much water vapour is present in steam. It is denoted by ‘x’.
Where M=mass of the dry steam
m=mass of water vapour
The uses of dryness fraction allow us to know both the mass of dry steam and mass of water vapour.
Now, see
If that means dry steam is 0.9 kg and water vapour is 0.1 kg in 1 kg of given steam.
Obviously for dry steam,
Quality is represented in percentage but meaning is same as ‘x’. If quality of steam is 80%, then it has 80% of dry steam and 20 % water vapour by mass.
(2) Specific volume:
Gases (steam is a gas) occupy less space under higher pressure than under lower pressure. This means 1 kilogram of steam occupies different volumes, depending upon its pressure. The term specific volume refers to the volume that one kg of steam occupies at a given pressure and temperature.
(3) Enthalpy
The total heat content of a substance is called enthalpy. Actually it has much broad definition in thermodynamics but for 1st year BME students this definition works just fine. So, total heat content by steam is termed as its enthalpy. It is denoted by ‘H’. SI unit is KJ
‘h‘ is generally used term which represents specific enthalpy, unit for which is KJ/Kg.
The enthalpy of liquid that is water at boiling point that’s why subscript ‘l’ is used, point ‘D’ in h-T diagram. Similarly is enthalpy of dry saturated steam, point ‘E’ in h-T diagram and is the latent heat, Process D to E in h-T diagram.
Different types of boilers
A steam boiler or steam generator is a closed vessel in which water is heated, vaporized and converted into steam at a pressure higher than atmospheric pressure. Boiler is a closed vessel in which heat produced by combustion of fuel is transferred to water for its conversion in to steam at the desired temperature and pressure. According to IBR a boiler is closed pressure vessel with capacity exceeding 25.75 litres used for IBR a boiler is closed pressure vessel with capacity exceeding 25.75 Litres used for generating steam under pressure it includes all the mountings fitted to such vessels which remains wholly or partly under pressure when steam is shut off.
Working Principle of a Boiler
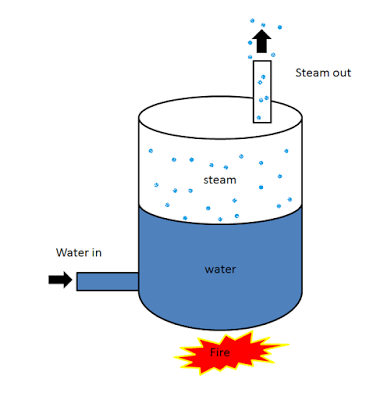
The boiler works on the same principle as the water is heated in a closed vessel and due to heating, the water changes into steam. This steam possesses high-pressure kinetic energy. The boiler contains water. The water is heated to its boiling temperature by the use of heat from the furnace. Due to the heating of water, it gets converted into high-pressure steam. The steam generated is passed through the steam turbines. As the high-pressure steam strikes the turbine, it rotates the turbine. A generator is attached to the turbine and the generator also starts to rotate with the turbine and produces electricity.
Classifications or Types of Boiler:
There is large number of boiler designs, but they may be classified according to the following ways:
1) According to the circulation of gases:
- Fire-tube boiler
- Water-tube boiler
Fire Tube Boiler:
As it indicated from the name, the fire tube boiler consists of numbers of tubes through which hot gasses are passed. These hot gas tubes are immersed into water, in a closed vessel. Actually in fire tube boiler one closed vessel or shell contains water, through which hot tubes are passed. These fire tubes or hot gas tubes heated up the water and convert the water into steam and the steam remains in same vessel. As the water and steam both are in same vessel a fire tube boiler cannot produce steam at very high pressure. Generally it can produce maximum 17.5 kg/cm2 and with a capacity of 9 Metric Ton of steam per hour.
(a) Hot gases formed after the combustion of fuel flow through tubes and water surrounds these tubes.
(b) Internally fired.
(c) Working pressure limited to 20 bars.
(d) Steam generation rate is lower.
(e) For a given power, it occupies large floor area.
(f) Not suitable for large power plants.
(g) It carries lot of risk on less working pressure.
(h) Different parts cannot be separated easily, hence it becomes difficult to transport.
(i) Water treatment is not necessary.
(j) Parts are not so accessible for cleaning and inspection.
Fire tube boilers are classified as follows.
l. External furnace:
(i) Horizontal return tubular
(ii) Short fire box
(iii) Compact.
2. Internal furnace:
(i) Horizontal tubular
(a) Short firebox (b) Locomotive (c) Compact (d) Scotch.
(ii) Vertical tubular.
(a) Straight vertical shell, vertical tube
(b) Cochran (vertical shell) horizontal tube.
Advantages of Fire Tube Boiler
- It is quite compact in construction.
- Fluctuation of steam demand can be met easily.
- It is also quite cheap.
Disadvantages of Fire Tube Boiler
- As the water required for operation of the boiler is quite large, it requires long time for rising steam at desired pressure.
- As the water and steam are in same vessel the very high pressure of steam is not possible.
- The steam received from fire tube boiler is not very dry.
Water Tube Boiler:
A water tube boiler is such kind of boiler where the water is heated inside tubes and the hot gasses surround them.
This is the basic definition of water tube boiler. Actually this boiler is just opposite of fire tube boiler where hot gasses are passed through tubes which are surrounded by water.
(a) Water flows inside the tubes and gas surrounds these tubes.
(b) Externally fired.
(c) Working pressure may be as high as 150 bars.
(d) Steam generation rate is higher.
(e) For a given power, it occupies lesser floor area.
(f) Suitable for large power plants
(g) Less risk on explosion due to high working pressure.
(h) Each and every part can be separated easily, so transportation is easier.
(i) In this boiler, water treatment is necessary.
(j) Parts are easily accessible for inspection.
There are many types of water tube boilers, such as
- Horizontal Straight Tube Boiler.
- Bent Tube Boiler.
- Cyclone Fired Boiler.
Horizontal Straight Tube Boiler again can be sub-divided into two different types,
- Longitudinal Drum Water Tube Boiler.
- Cross Drum Water Tube Boiler.
Bent Tube Boiler also can be sub divided into four different types,
- Two Drum Bent Tube Boiler.
- Three Drum Bent Tube Boiler.
- Low Head Three Drum Bent Tube Boiler.
- Four Drum Bent Tube Boiler.
Advantages of Water Tube Boiler
There are many advantages of water tube boiler due to which these types of boiler are essentially used in large thermal power plant.
- Larger heating surface can be achieved by using more numbers of water tubes.
- Due to convectional flow, movement of water is much faster than that of fire tube boiler; hence rate of heat transfer is high which results into higher efficiency.
- Very high pressure in order of 140 kg/cm2 can be obtained smoothly.
Disadvantages of Water Tube Boiler
- The main disadvantage of water tube boiler is that it is not compact in construction.
- Its cost is not cheap.
- Size is a difficulty for transportation and construction.
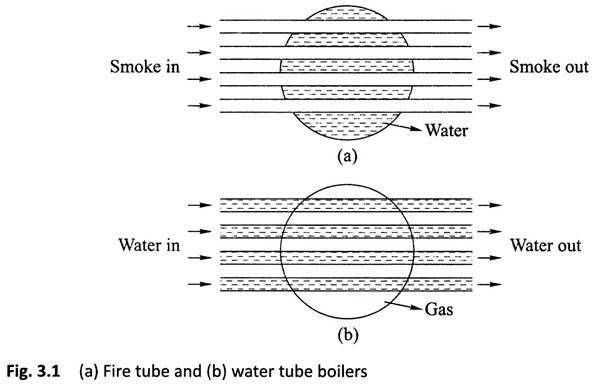
2) According to Circulation of water:
- Free circulation
- Forced circulation
Free circulation:
In any water heating vessel heat is transmitted from one place to another not by condition but by convection because water is a bad conductor of heat.Let vessel containing water be heated at its bottom, as the water in the bottom portion is heated therefore its density becomes reduced in comparison to the density of water in the upper portion of the vessel, as a result, the less dense water at the bottom portion of the vessel rise up and comparatively more dense and cold water at the upper portion of the vessel comes down to take its place and thus a convection current is set up in the water until temperature off all water becomes the same.
The method of circulation of water described above is known as free circulation.
In boilers like Lancashire, Babcock, and Wilcox, etc. free circulation of water takes place.
- Advantages of free circulation:
The advantages of free circulation are:
- Free circulation of water helps to maintain a uniform temperature true everywhere within the boiler so that unequal expansion of various parts of the boiler is prevented.
- Free circulation of water facilities the escape of steam from the heating surface as soon as it formed. If steam does not escape quickly after its formation the boilerplates do not remain constantly in touch with water and as a result, these plates may be overheated.
Forced Circulation:
In forced circulation, pumps are used to maintains the continuous flow of water in the boiler. In such a case, the circulation of water takes place due to pressure created by the pump.
The forced circulation system is adopted in more high pressure, high capacity boilers of all of which are water tube type boiler.
- Advantages of forced circulation:
The advantages of forced circulation are:
- The rate of heat transfer from the flue gases to the water higher.
- Tubes having comparatively smaller diameters can be used. This reduces the overall weight of the boiler.
- The number of boiler drums required may be reduced.
- Less scale formation in the boilers is required.
- Steam can be quickly generated.
- The fluctuation of load can be easily met without taking the help of any complicated controlled device.
- Chance of overheating of the boilerplates in minimum.
- Weight per unit mass of steam generated is less.
3) According to the number of tubes used:
According to the number of tubes, Boilers may be classified as:
- Single tube boiler
- Multi-tube boiler
Single tube boiler:
The boiler having only one fire tube or water tube is called single tube boiler.
Example: Cornish boiler
Multi tube boiler
The boiler having two or more fire or water tubes for the circulation of hot gases or water are called multi tube boiler.
Example: Lancashire boiler, Locomotive boiler, Cochran boiler, Babcock and Wilcox boiler etc.
4) According to the nature of use:
According to nature use, boilers are classified as
- Stationary boilers
- Locomotive boilers
- Marine boilers.
Stationary boilers:
For the generation of thermal power and for process work (in chemical, sager and textile industries) boilers used are called stationary boiler.
Locomotive boilers:
Boilers used in locomotive steam engines are called locomotive boilers.
Marine boilers:
Boilers used in steamships are called marine boilers.
5) According to the nature of the fuels used:
According to the nature of the fuel used boiler may be:
- Fuel-fired
- Gas fired
- Liquid fuel fired
- Electrically fired
- Nuclear fired
NOTE: Babcock and Wilcox boilers use solid or gaseous fuel.
Volex boilers use oil fuel.
6) According to the pressure of the boiler:
- High-pressure boiler
- Medium-pressure boiler
- Low-pressure boiler
High-pressure boiler:
The pressure of the boiler above 80 bar.
Medium-pressure boiler:
It has a working pressure of steam from 20 bar to 80 bar. It is used for power generation or process heating.
Low-pressure boiler:
This type of boiler produces steam at 15-20 bar pressure. This is used for process heating.
7) According to the position of the axis of the boiler shell:
According to the position of the axis of the boiler shell, boilers are classified as:
- Vertical boiler
- Horizontal boiler
Vertical boiler:
If the boiler axis is vertical, it is called a vertical boiler. For example, Cochran boiler.
Horizontal boiler:
If the boiler axis is horizontal, it is called a horizontal boiler.
For example, Lancashire boiler.
8) Stationary boiler and Portable boiler
a) Stationary boilers: The boilers which cannot be transported easily from one place to another are called stationary boilers.
Example: Lancashire boiler, Babcock and Wilcox boiler
(b) Portable boiler: The boilers which can be easily transported (moved) from one place to another are called portable boilers.
Example: Locomotive boiler.
9) Externally tired and internally tired boilers
(a) Externally fired boiler: - In the boiler if the fire is outside the shell, that boiler is known as externally fired boiler. Example: Babcock & Wilcox boiler
(b) Internally fire boiler:- In the boiler in which the furnace is located inside the boiler shell it is known as internally fired boiler. Example: Cochran boiler, Lancashire boiler etc.
Boiler mountings and accessories
The boiler mountings are fittings which are mounted on the boiler for its proper functioning. Mountings are water level indicator, safety valve, pressure gauge, etc. It may be noted that a boiler cannot function safely without the mountings.
Following are the important boiler mountings:
- Water level indicator
- Pressure gauge
- Safety valve
- Stop valve
- Blow off
- Feed check valve
- Fusible plug
1) Water Level Indicator
It is an main fitting in the boiler, Water level indicator indicates the water level inside the boiler. It is a safety device upon which safe working of the boiler depends.
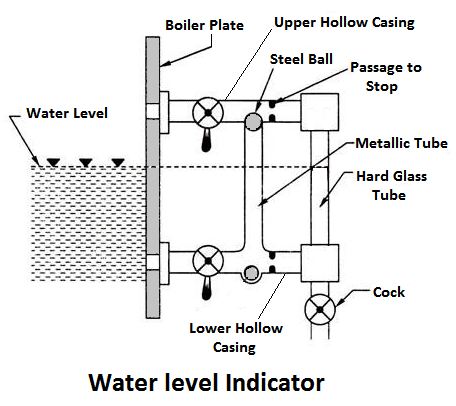
Working Principle
Water-tube indicator the water consists of a vertical hard glass tube G which is fitted with two gunmetal tubes A and B. The tubes A connects the steam space of the boiler with the glass tube and the tube B connects the water space of the boiler with the glass tube.
The tube A is provided with a valve ‘S’, called a steam valve, and tube B is fitted with another valve ‘W’, called a water valve. In addition to these valves, a third valveD, called drain valve, is fitted to the water level indicator through which water together with condensed steam from the gunmetal tube A is drained from time to time.
2) Pressure Gauge
Pressure gauges are used to measure the pressure of steam inside a steam boiler. The pressure gauge is fixed in front of a steam boiler.
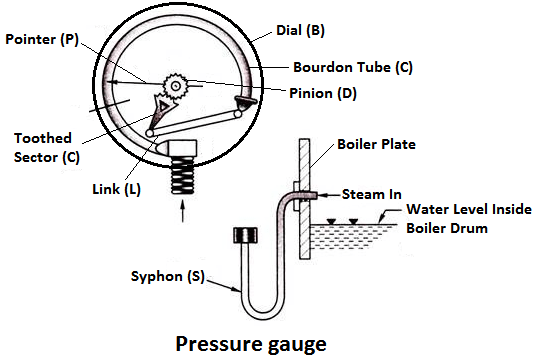
Working Principle
The pressure gauge shown in fig is bourdon pressure gauge. It consists of a circular spring tube A. One end of the bourdon tube is closed and connected to a link L and the other end is squared to a Hollow block B. The link L connects the closed end of the tube to the toothed sector C which is hinged at O. The toothed sector gears with pinion D which carries a pointer P. The pointer moves on a dial graduated in pressure units.
3) Safety valves
These are the devices attached in the steam boiler for preventing explosions due to excessive internal pressure of steam.
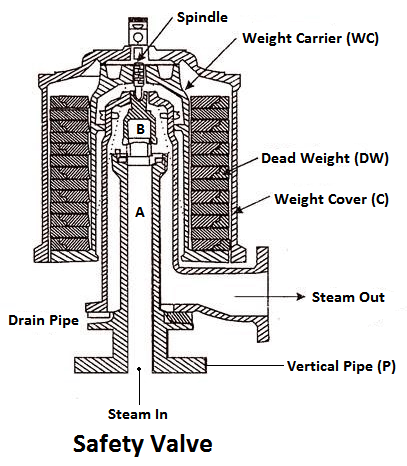
The safety valve is an instrument which prevents the boiler pressure from rising above its normal working pressure by automatically opening when the boiler pressure exceeds the normal working pressure, Thus allowing excess steam to escape into the atmosphere until the pressure comes down to its normal valve. Thus, a safety valve ensures safety to a boiler from being damaged due to excessive steam pressure.
The safety valves commonly used are:
- Deadweight safety valve,
- Lever safety valve,
- Spring-loaded safety valve,
4) Steam stop valve
The function of a stop valve is to control the flow of the steam from within the boiler and to stop it completely when required. A stop valve or junction valve is used to regulate the flow of steam from the boiler.
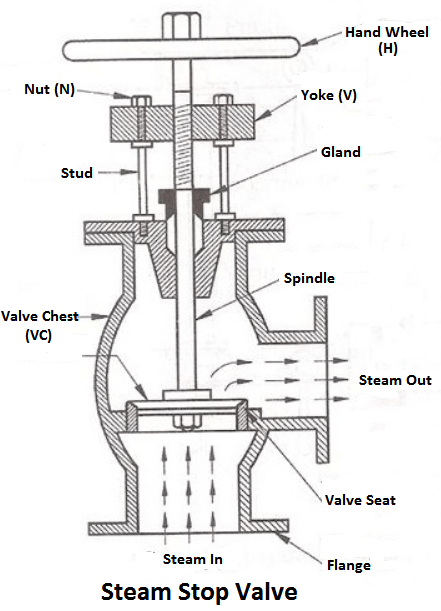
The valves mounted on the boilers, which change the direction of flow of steam by 90° are called junction valves, while valves fitted in pipelines which allowing the steam in the same direction are called stop valve.
5) Blow-off Valve
The function of a blow-off valve is to remove periodically the sediments deposited at the bottom of the boiler while the boiler is in operation and to empty the boiler while it is being cleaned or inspected.
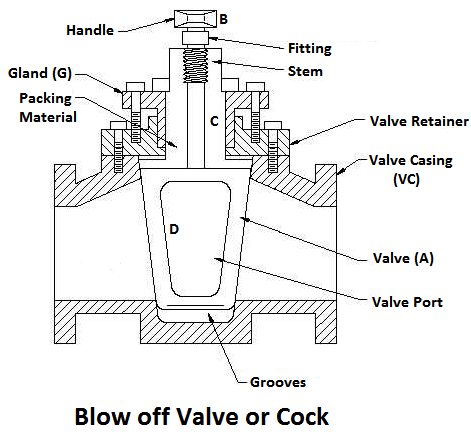
When the blow-off valve is opened the water which is under the pressure of steam, rushes out with tremendous velocity thus carrying out the sediments along with it.
6) Feed Check Valve
When the level of water in the boiler falls, it is brought back to the specified level by supplying the additional water called feed water. The pressure inside the boiler will be high therefore the pressure of the feed water has to be raised by a pump before it is fed into the boiler. The feed water under high pressure is fed into the boiler through the feed check valve.
The function of a feed check valve is to control the flow of water from the feed pump to the boiler and to prevent the backflow of water from the boiler to the pump when the pump pressure is less than the pressure or when the feed pump ceases to work. Evidently feed check valve is placed at the boiler end of the delivery pipe of the feed pump.
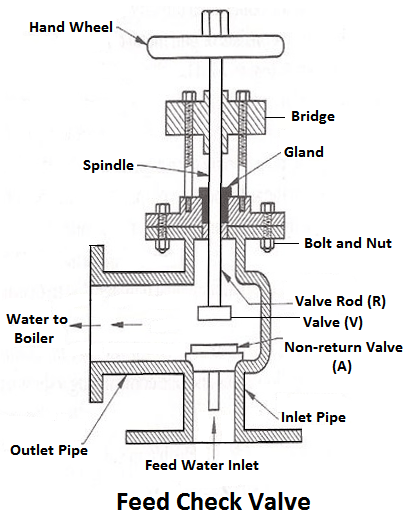
7) Fusible Plug
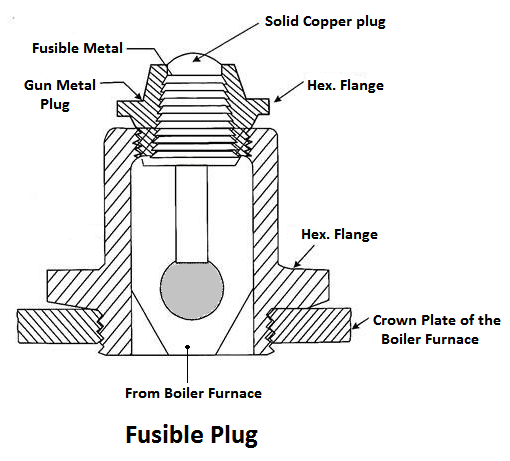
The plug is made up of tin or leads alloy, which has a low melting point. The function of the fusible plug is to put-off the fire in the furnace of the boiler when the water level falls below an unsafe level and thus avoids the explosion, which may take place due to overheating of the tubes and the shell. It is fitted over the crown of the furnace or the combustion chamber.
Boiler Accessories
The boiler accessories are required to improve the efficiency of the steam power plant and to enable for the proper working of the boiler. The boiler accessories are the devices, which form an integral part of a boiler but are not mounted on it. They include superheaters, economiser, feed pump etc. It may be noted the accessories help in controlling and running the boiler efficiently.The boiler accessories aren’t mounted directly on the boiler.
The essential boiler accessories are:
- Economiser
- Air pre-heater
- Superheaters
- Feed pump
- Steam Separator
- Steam trap
1) Economiser
The combustion gases coming out of the boiler contain a large quantity of heat. Therefore the maximum amount of heat from the gases should be recovered before it escapes to the chimney.
In the economiser, heating the feed water does the recovery of heat in the flue gases. The economiser is placed in the path of the gases. They improve the overall efficiency of the boiler by reducing fuel consumption.
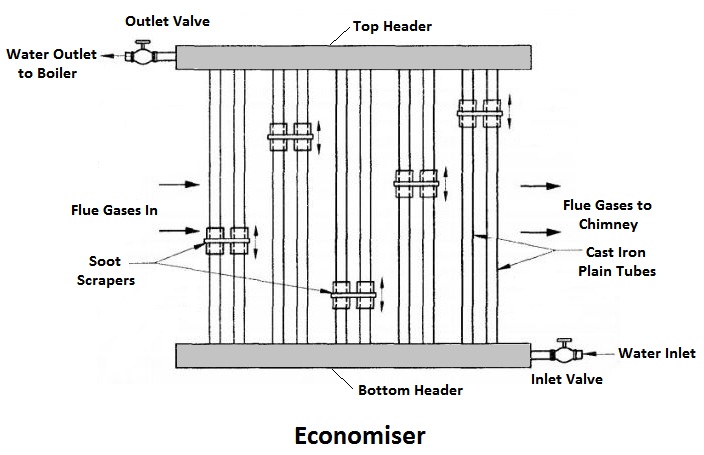
2) Air Pre-heater
The air preheater is an accessory that recovers the heat in the exhaust gas by heating the air supplied to the furnace of the boiler. Supplying preheated air into the furnace produces a high furnace temperature and accelerates the combustion of the fuel. Thus the thermal efficiency of the plant will be increased.
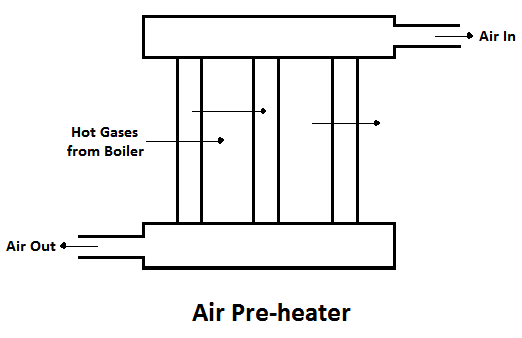
The advantages of air pre-heater are,
- Increase in the steam generation rate.
- Better combustion with less soot, smoke and ash, and
- Low-grade fuels can be used.
3) Superheaters
The superheaters are used in boilers to increase the temperature of the steam about the saturation temperature.
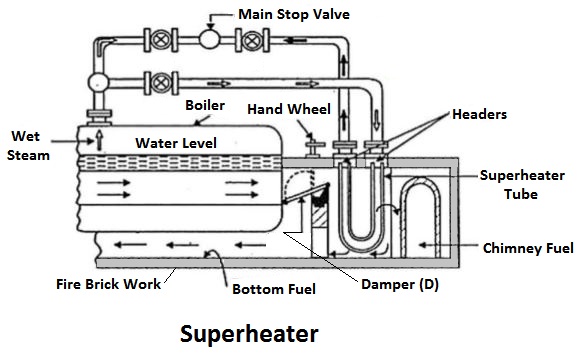
The dry saturated steam generated in the boiler is passed through a set of tubes placed in the path of the flue gases, in which it will be heated further by the hot gas to increase its temperature about the saturation temperature.
4) Feed Pump
A feed pump is a boiler accessory required to force the feed water at high pressure into the boiler. Commonly used pumps are,
- Reciprocating pumps
- Rotary pumps
The reciprocating pumps are driven directly by coupling them to the steam engine. The rotary pumps are driven by the steam turbines or by electric motors.
Formation of Steam:
Generation of One Kg of Steam at a Given Pressure from Water Initially At 0°C:
Let us decide upon the pressure under which one kg of water is to be heated. After fixing the pressure we can know the saturation temperature for this pressure from steam tables and thus, water should be heated to this temperature before steam will be generated.
Fig.Illustrates the three stages in the formation of steam, at some constant pressure, namely:
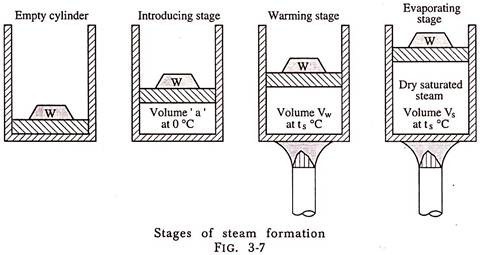
(1) Introducing Stage:
During this stage 1 kg of water at 0°C is pumped into the cylinder against an absolute pressure P, the pressure of steam generation. This pressure is caused by the weight placed on piston and pressure of atmosphere. The energy expended by the pump to deliver 1 kg of water of volume ‘a’ m3 at 0°C equals P × a (joules). This energy appears as pressure energy of the water in the cylinder and could be made to do work by virtue of its pressure.
(2) Warming Stage:
During this stage heat is added to the water so that its temperature from 0°C is raised to ts (the temperature at which steam will begin to form under absolute pressure P) and to increase the volume of 1 kg of water from ‘a’ to vf, where vf is the volume of 1 kg of water at the temperature ts and absolute pressure P. The heat supplied during this warming stage is called Sensible Heat because it can be detected by the sense of touch and produces a rise in temperature (ts – 0)°C to be seen on a thermometer.
The sensible heat supplied in the warming stage is used for two purposes:
(i) To increase the temperature of 1 kg of water from 0°C to ts. This heat is utilized for increasing the internal energy of water.
(ii) To do external work dw (vf – a) Joules in increasing the volume of water from ‘a’ to vf against the absolute pressure P which acts constantly on the piston.
By First Law of Thermodynamics as applied to non-flow process, heat supplied = change in internal energy + external work done by water.
Sensible heat = hf — (uf – uo) + P (vf– a).
If the changes in internal energy are reckoned from 0°C, u0 will be zero and we get sensible heat-
Hf = uf + P (vf – a).
(3) Evaporating Stage:
During this stage further heat is supplied to 1 kg of water at tsat, the saturation temperature corresponding to the constant pressure P. The volume changes from vf to vg. During this stage heat is added at constant temperature and this heat is utilized in changing the state of water. The external work done during this stage = P (vg – vf).Because the heat added during this stage cannot be recorded by a rise in temperature on the thermometer, it is called Latent Heat (hidden heat) or heat of vaporization i.e., hfg.
The latent heat is, therefore, used for two purposes:
(i) To overcome the internal molecular resistance of the water by changing it from water into steam.
(ii) To force back the piston to increase the volume from that of water to that of steam. By First Law of Thermodynamics as applied to non-flow process, heat supplied = change in internal energy + external work done.
Heat of vaporization = hfg = (ug– uf) + P (vg –vf).
Thus, we see that energy is supplied in three stages to generate steam at a pressure P from water at 0°C.
Conditions of Steam:
Steam may occur in any one of the following three conditions:
(i) Saturated steam which may be either dry or wet
(ii) Superheated steam
(iii) Supersaturated steam.
i. Saturated Steam:
Saturated steam is any steam which cannot have heat abstracted from it or be compressed at constant temperature without partially condensing. Saturated steam is a vapour at the temperature corresponding to the boiling point of the liquid at the imposed pressure. We have seen that for each liquid, a certain definite boiling point exists for each pressure.
A substance which is in the vapour state in a confined space and is in contact with some of the same substance in liquid state is always at the same temperature as the liquid and is saturated vapour. When heat is applied to saturated vapour and to the liquid with which it is in contact, more liquid evaporates but the temperature remains constant. Similarly if heat is removed more of the vapour condenses but the temperature will remain constant.
Dry Saturated Steam and Wet Steam:
If saturated steam does not contain any water, it is known as Dry Saturated Steam. It contains just sufficient heat energy to maintain all of the water in a gaseous state. If saturated steam contains liquid particles, it is known as Wet Steam.
It does not contain sufficient heat energy to maintain all water in the gaseous state. If some of the heat energy is absorbed from the dry saturated steam, the steam becomes wet. In practice it is difficult to get an absolutely dry saturated steam if it is produced by boiling, because some of water particles are carried away with the steam.
Ii. Superheated Steam:
If the temperature of the steam is greater than that of the boiling point corresponding to the pressure of steam generation, the steam is known as Superheated Steam. The temperature of the steam may be increased above the saturation temperature by adding heat to steam, after all the water has been vaporized or after the steam has been separated from contact with water.
If superheated steam is brought in contact with water it will give up part of its heat to the water. If the superheated steam contains sufficient heat energy, in that case, the water will evaporate. If superheated steam does not contain sufficient heat to evaporate all the water into steam, it will give up all its additional heat known as superheat and some of the water will not be converted into steam.
The amount of superheat in steam is given in terms of the difference between its temperature and that of the saturated steam at the pressure of steam generation. If the temperature of steam is 100°C higher than the saturation temperature corresponding to the pressure of steam generation, we say that the steam has 100°C of superheat.
Iii. Supersaturated Steam:
Supersaturated steam at a particular saturation pressure has temperature less and density greater than the corresponding values given in steam tables. This condition of steam is obtained when it is cooled by its own expansion until it contains less heat energy than the saturated steam under the same conditions. This state of steam is very unstable and the steam soon resumes the saturated condition; such a state of steam occurs in expansion in a nozzle.
Properties of Steam:
The properties of steam are interrelated. If we know certain properties, the other properties may be found out. For example, if the pressure of saturated steam is observed by the pressure gauge, its temperature can be found from steam tables in which the results of various experiments have been tabulated. All other properties of a given mass of steam can be known when any two properties such as the pressure and dryness fraction of steam for saturated steam and pressure and degree of superheat for superheated steam are known.
If the steam is dry and saturated then only pressure should be known to determine all the properties. Thus, in order to observe the above two properties of steam, pressure gauges and steam calorimeters or thermometers are used at suitable points.
How Does a Steam Turbine Work
In simple terms, a steam turbine works by using a heat source (gas, coal, nuclear, solar) to heat water to extremely high temperatures until it is converted into steam. As that steam flows past a turbine’s spinning blades, the steam expands and cools. The potential energy of the steam is thus turned into kinetic energy in the rotating turbine’s blades. Because steam turbines generate rotary motion, they’re particularly suited for driving electrical generators for electrical power generation. The turbines are connected to a generator with an axle, which in turn produces energy via a magnetic field that produces an electric current.